Gene-Editing Tools: Delivery Methods and Challenges
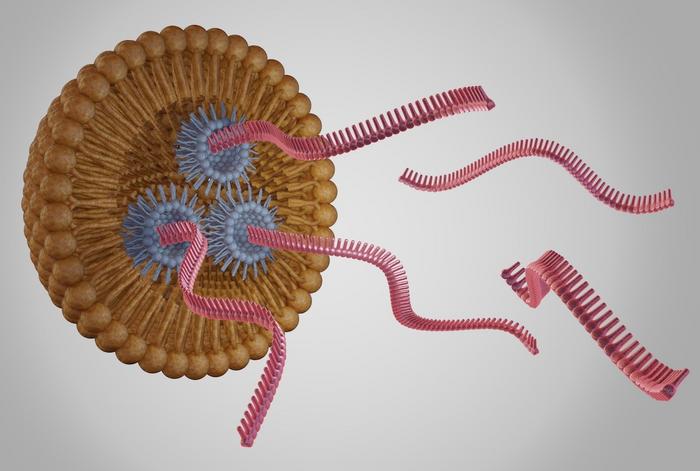
Gene editing with CRISPR-Cas9 emerged in 2012 as a revolutionary tool for treating and preventing a broad range of genetic, degenerative, metabolic, and infectious diseases. Several related technologies have been developed since then, including base editing, prime editing, epigenome editing, and various other Cas nucleases have emerged. All CRISPR-based gene-editing modalities rely on at least a Cas nuclease and a guide RNA (gRNA), but in some workflows, additional reagents, for example, additional gRNAs, a DNA template or Cas9-fusion proteins, are required. Gene-editing tools can be delivered in three formats: mRNA, DNA or as a ribonucleoprotein (RNP) complex.
In all cases, gene-editing tools must be delivered to the desired cell type to ensure high gene-editing efficiency only at the target sequence and without unwanted immune responses or other adverse effects. All these parameters are impacted by how the tools are delivered, so the mode of delivery is of paramount concern when gene editing is used therapeutically. Whether the gene-editing strategy is in vivo or ex vivo, researchers currently have two main approaches to choose from, namely viral and non-viral delivery systems.
It's important to note that the choice between viral and non-viral methods depends on various factors, including the specific application, target tissue, size of the genetic material being delivered, and safety considerations. The landscape of therapeutic gene editing is rapidly evolving, and the prevalence of these methods in clinical trials may change as new technologies and delivery systems are developed.
CRISPR Medicine News (CMN) has spoken with multiple experts in gene editing about the advantages and limitations of different delivery methods. In this article, we will discuss the benefits and limitations of the available technologies. Though we acknowledge that most gene-editing therapeutic candidates being evaluated in ongoing clinical trials use viral delivery, we will only deal briefly with that approach. Instead, we will focus on non-viral delivery systems since these are gaining significant momentum and increasingly advancing into clinical trials.
Viral delivery systems
Viruses are designed by nature to deliver genetic material to cells, which is reflected in the high efficiency of viral vectors to deliver gene-editing tools. Adenovirus (AdV), lentivirus (LV), and adeno-associated virus (AAV) are all viral vectors commonly used in gene therapy, including CRISPR-Cas and related gene-editing applications. Each vector has distinct characteristics that influence its suitability for specific applications.
AdVs are large, non-integrating viruses capable of carrying substantial genetic payloads, up to 37 kb. They can infect many cell types, including non-dividing cells, making them versatile for gene therapy. However, their significant drawback is the potential to trigger strong immune responses, limiting their effectiveness and posing safety concerns.
LVs, a subclass of retroviruses, can integrate their genetic material into the host genome. This makes them useful for traditional gene therapy, where a functional copy of a missing or mutated gene is introduced. However, LV integration is often undesirable in gene-editing therapies because the prolonged transgene expression (of the introduced gene-editing reagents) increases the risk of off-target editing and raises concerns about insertional mutagenesis. LVs are more suited for targeting dividing cells, which may limit their use in certain types of tissues.
LV-based delivery of CRISPR tools made headlines in December 2022 when the approach was used to treat and cure a 13-year-old girl who had relapsed T-cell acute lymphoblastic leukaemia (T-ALL) as part of a Phase 1 clinical trial of BE-CAR7. Here, LV delivery was used ex vivo to express a chimeric antigen receptor (CAR) in T cells that were subsequently base edited to make them evade the immune system in an allogenic host (1).
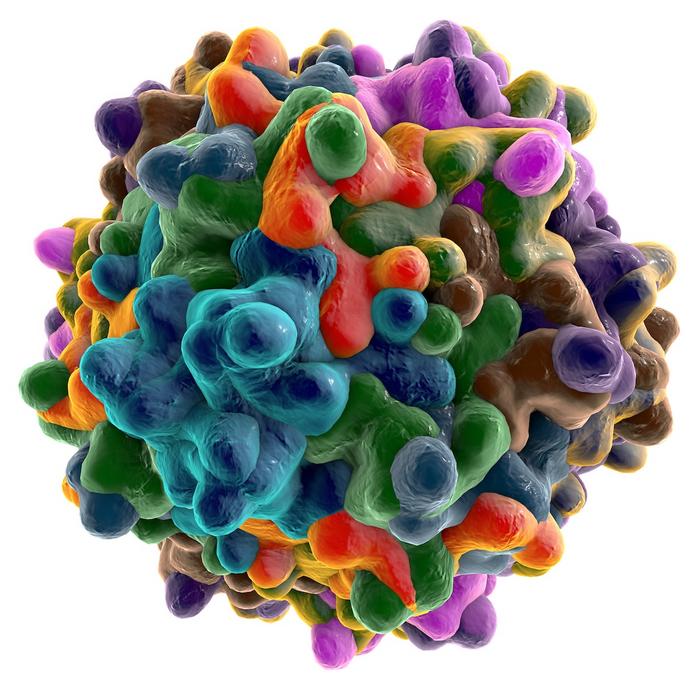
On the other hand, AAVs are smaller and less immunogenic than AdVs, and unlike LVs, they do not integrate into the host genome, reducing the risk of insertional mutagenesis. AAVs can infect both dividing and non-dividing cells, broadening their applicability. Similarly to AdVs, however, they can carry only about 8 kb, which can be a significant limitation for gene-editing applications using base- and prime editors. AAVs are often preferred for in vivo applications due to their lower immunogenicity and safety profile.
Current clinical-stage gene-editing programmes that use AAV-based delivery include therapeutic candidates for conditions like the inherited eye disorder Leber congenital amaurosis type 10 (LCA10), Duchenne muscular dystrophy (DMD) and HIV. We recently interviewed the CEO and Founder of Siren Biotechnology, Nicole Paulk, who is an expert in AAV delivery. Paulk said that she expects to see a significant increase in gene-editing AAV therapies, going from one approval every couple of years, to handfuls and then dozens of approvals per year. This is primarily due to the recent elimination of major bottlenecks in the production of AAV and the engineering of AAV capsids that target specific tissues beyond the liver, brain, eye and skeletal muscle.
Physical, non-viral delivery systems
Non-viral delivery technologies can be divided into physical and chemical methods. The most common physical method is electroporation, which applies electrical pulses to open pores in the cell membrane through which gene-editing tools can enter. Electroporation can only be used on isolated cells in the laboratory and is thus restricted to in vitro and ex vivo applications.
Electroporation is suitable for most cell types, including primary cells, and allows for large cargo delivery. It is, however, stressful for cells and may lead to a high percentage of cell death. Electroporation has been used in the development of a number of clinical-stage ex vivo gene-editing therapeutic candidates, e.g., to treat beta-thalassemia and sickle cell disease, blood cancers and solid tumours.
Nanoparticles challenge viruses
In contrast to the more traditional viral methods, non-viral delivery vehicles are emerging as promising alternatives. These include lipid nanoparticles (LNPs) with a single lipid layer that surrounds a lipophilic interior, in which the payload is encapsulated in smaller, inverted LNPs with a lipophilic exterior and a hydrophilic interior. Liposomes, on the other hand, comprise a lipid bilayer surrounding an aqueous pocket. Extracellular vesicles (EVs) are naturally occurring, bilayered structures secreted by various cells, which transport diverse bioactive molecules, including exosomes, and they are purified from serum before being loaded with gene-editing reagents.
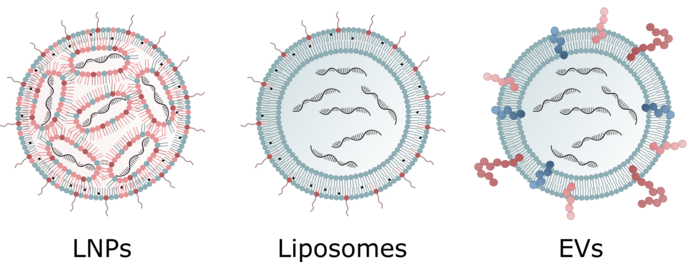
LNPs - known for their high loading efficiency, flexible design, and biocompatibility - have been successfully used in the development of RNA-based COVID-19 vaccines, and are currently used in several clinical-stage gene-editing therapies. However, challenges such as rapid blood clearance, liver accumulation, and endosomal degradation susceptibility can lower the gene-editing efficiency of LNPs.
Several clinical trials use LNPs for the delivery of therapeutic gene-editing tools to treat in vivo, for example, Verve Therapeutics’ ongoing Phase 1b trial of VERVE-101 for familial hypercholesterolemia, Intellia Therapeutic’s Phase 3 trial of NTLA-2001 for transthyretin amyloidosis (ATTR), and its Phase 1/2 trial of NCT05120830 for hereditary angioedema (HAE). A number of research studies have explored the potential of LNP-based gene-editing therapy for other diseases, for example in animal models of ovarian cancer and glioblastoma (2) and hypolipidemia (3).
Dan Peer is the director of the Laboratory of Precision NanoMedicine at Tel Aviv University, and his team is developing LNPs for the safe and efficient delivery of Cas9 mRNA and sgRNAs to treat aggressive cancers. »We've made significant progress, especially with targeted delivery for haematological malignancies and solid tumours using mRNA that produces Cas9. Achieving up to 95% genome editing in vivo in a cell-specific manner that is translated to almost curative effect is unprecedented,« he says.
LNPs comprise a complex and critical mixture of lipids, usually including an ionisable lipid and several structural lipids. Ionisable lipids remain neutral at physiological pH but become positively charged at low pH in the endosomes, and this feature promotes endosomal escape and reduces the toxicity of the LNPs.
LNPs offer three main advantages that provide a faster path to the clinic, explains Peer: »Firstly, there are many suitable ionisable lipids available, and they can be scaled up quickly. Secondly, it's relatively easy to produce good manufacturing practice (GMP)-grade materials for clinical trials, and regulatory agencies are familiar with LNPs, making the approval process smoother. And thirdly, some lipids are fully degradable, thermostable, and can encapsulate large payloads, which viral vectors might struggle with«.
“How you orient your targeting moiety, for example, an antibody, can dramatically impact efficacy and toxicity”Dan Peer
According to Peer, the main limitation of LNPs is that they can't provide sustained release but instead exhibit a burst release pattern. There's no slow-release option, which might be preferred for some diseases. Another downside is that LNPs and other nanoparticles get hijacked by the liver. If targeting blood cells, you might have more success before the LNPs reach the liver, but for other organs or solid tumours, the liver often captures a significant portion,« he says.
Add-ons target nanoparticles to specific tissues
Various ligands or antibodies can be incorporated into the lipid layer so the nanoparticles bind to target cells, and this can reduce accumulation in the liver. However, this is a challenging task. Peer explains: »How you orient your targeting moiety, for example, an antibody, can dramatically impact efficacy and toxicity. If the targeted system isn't accurately conjugated, it might expose the Fc region of an antibody and be rapidly taken up by circulating leukocytes and phagocytes with Fc receptors.«
In 2020, Peer’s team succeeded in using antibody-guided LNPs to target the epidermal growth factor receptor (EGFR) that is abundantly expressed in ovarian cancer cells (2). Those experiments were carried out in mice, and Anna Gutkin, who was then a PhD student in Peer’s lab and the study’s co-first author, explained to CMN:
»The survival after metastatic ovarian cancer increased by 80% with double injections. This has a significant impact on minimising the treatment regimen and improving patients' quality of life,« referring to the study’s potential clinical impact.
Daniel Siegwart at the University of Texas Southwestern Medical Center has taken another approach with specialised nanoparticles that can deliver CRISPR cargo to various specific organs and tissues. He has developed the SORT system, where particular lipids are added to LNPs in carefully balanced proportions, altering their tissue tropism (4). » Similar to very low-density lipoprotein (VLDL) particles in our blood, SORT LNPs adsorb proteins from the plasma that mediate uptake in specific cells. We are currently working on identifying new SORT molecules to enable delivery enrichment in additional tissues,« says Siegwart.
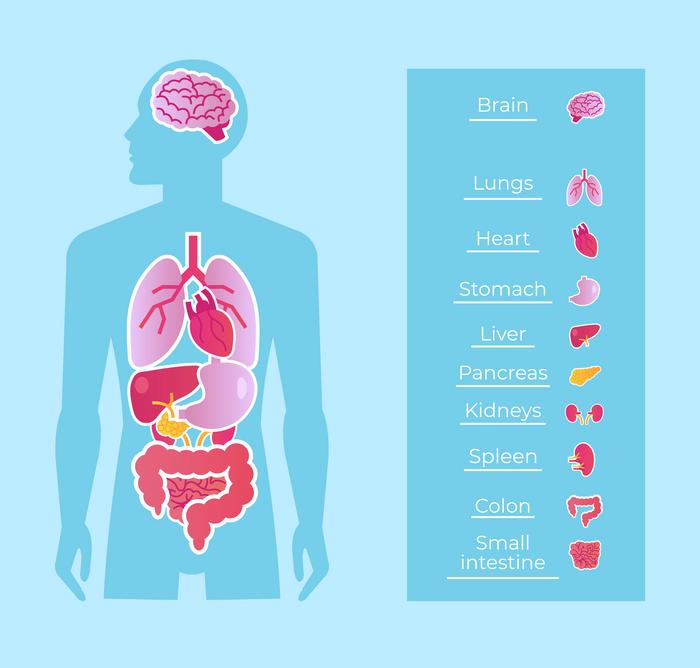
Siegwart told us in a previous interview that SORT enables liver-, lung- and spleen-targeted CRISPR-Cas gene editing, and the technology is now licensed to California-based ReCode Therapeutics that is co-founded by Siegwart.
Another example of commercial utilisation of targeted LNPs is seen with Verve Therapeutics’ product candidate VERVE-102, which, according to a recent press release, is expected to enter a Phase 1 clinical trial in the first half of 2024. VERVE-102 follows the same therapeutic strategy as VERVE-101, which is currently being evaluated in Phase 1 clinical trial for lowering LDL cholesterol (LDL-C) levels in the blood of patients with high-risk heterozygous familial hypercholesterolemia (HeFH). Both candidates are based on in vivo base editing to permanently disrupt the PCSK9 gene in the liver.
VERVE-101 uses non-targeting LNPs and has shown encouraging preliminary results, achieving a sustained 50% reduction in LDL-C. VERVE-102, however, uses LNPs with the company’s proprietary GalNAc-based ligand, and pre-clinical experiments in non-human primates show that this modification can increase base editing in the liver from 5% to 61% with minimal editing in other tissues (5). These results suggest that targeting LNPs can improve the company's already promising efforts to lower LDL-C in patients.
Dedicated delivery strategies have also been developed to target the brain, which poses a unique challenge since this delicate organ is protected by the blood-brain barrier (BBB), a highly selective semi-permeable border of endothelial cells. In 2022, Bingyang Shi at Macquarie University in Sydney decorated nanocapsules with angiopep-2 peptide - a ligand that binds low-density lipoprotein receptor–related protein-1 (LRP-1), which is more highly expressed on BBB endothelial cells and glioblastoma (GBM) cells.
In a previous interview with CMN, Shi explained how that ligand causes the nanocapsules to concentrate around the BBB, increasing their chance of penetration into the brain, where they will subsequently be preferentially taken up by GBM cancer cells. In vivo experiments in mice showed that targeting nanocapsules with the angiopep-2 peptide allowed them to cross the BBB more effectively, and they were taken up in GBM tumour tissue significantly better than non-targeting nanocapsules (6).
Guilherme Baldo, who is an associate professor at the Federal University of Rio Grande do Sul in Brazil, reported another successful attempt to cross the BBB without using any targeting moiety. In a previous interview with CMN in 2022, he explained: »Our main question was, how can we address the central nervous system without invasive approaches like direct injection into the brain? So, we came up with this idea of going through the nose to reach the olfactory bulb right above it.«
In that study, liposomes were loaded with CRISPR-Cas9 designed to treat a murine model of mucopolysaccharidosis I (MPS I) by knocking in a functional ɑ-L-iduronidase (IDUA) gene to compensate for the mutated copy in experimental mice (7). One-month-old mice received daily nasal administration (into each nostril) of the liposomal complex loaded with Cas9, gRNA and IDUA for 30 days, and measurements after 15 and 30 days showed a significant increase in serum IDUA activity compared to untreated mice.
»A new study is going to be performed in non-human primates, and this is expected to start next month [February 2024, ed.], in Brazil, « Baldo says.
Phages and bacteria deliver the tools to prokaryotes
Most gene-editing therapies are designed to target human eukaryotic cells directly (either ex vivo or in vivo). However, a handful of ongoing programmes aim to target the microbiome or infectious bacteria.
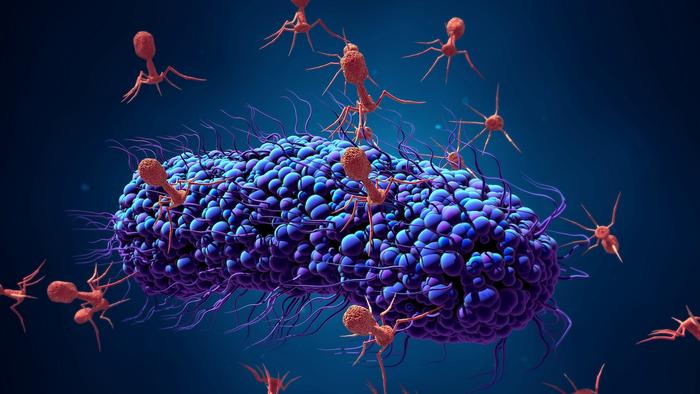
SNIPR Biome is a biotech company based in Copenhagen that has pioneered a clinical-stage CRISPR-based microbial gene therapy that uses CRISPR-armed phages to specifically target and remove pathogenic E. coli, including antibiotic-resistant strains, in the human gastrointestinal tract.
Senior scientist at SNIPR Biome, Yilmaz Emre Gençay, explains that they use a mixture of phages with engineered tail fibres loaded with CRISPR-Cas machinery – so-called CRISPR-armed phages. These modifications target the phages to infect and deliver their cargo specifically to the desired strains, leading to the cutting of the bacterial DNA and effectively killing the pathogen. In mice, the cocktail of CRISPR-armed phages, SNIPR001, led to a significant reduction of 4 log10 of a targeted E. coli strain (8). SNIPR001 has also recently been tested in a Phase 1 clinical trial, which demonstrated that SNIPR001 is safe and showed target engagement with E. coli in the gut of healthy humans.
»We used virulent phages capable of replicating once they find their host, but we can also use fully synthetic phages that we package with the desired DNA. These synthetic phages can't replicate, so they function solely for delivery, almost like an LNP,« Gençay says.
The company also uses proprietary delivery technology to enable in situ production and secretion of therapeutic proteins or peptides by delivering a therapeutic gene to the microbiome through living bacteria. Examples from the company’s pre-clinical pipeline include expression of enzymes, anti-cytokines and signalling hormones for treating gout, obesity and cardiometabolic diseases.
»Phages have a cargo size limit of around 12 KB, which is why we also use bacterial conjugation, where we don’t have these limitations. Both delivery methods are selective for prokaryotic cells and thus provide high safety,« Gençay adds.
Delivery by conjugation exploits the natural process of transferring a so-called conjugative plasmid between two bacterial cells in direct contact. Genetic elements encoding the Cas nuclease, gRNA and any other desired tools are inserted in a conjugative plasmid that is subsequently transformed into a suitable donor. When the donor is ingested or applied on the skin and comes into contact with a recipient in the microbiome, the tools are transferred to the recipient bacteria.
This process is highly efficient, says Professor Sébastien Rodrigue at Université de Sherbrooke in Québec who has been exploring various conjugation plasmids for several years: »We aim to match the delivery efficiency of phages, and several of our candidates succeed in this regard. For example, in the mouse gut microbiome, we can use conjugation to deliver CRISPR reagents and eliminate virtually all targeted antibiotic‐resistant E. coli bacteria.«
These remarkable results are achieved with a conjugative plasmid developed by accelerated laboratory evolution that uses adhesins for surface recognition to improve both specificity and duration of contact between the donor and recipient (9,10). The method has not been tested yet on humans but has shown promising results in piglets.
Rodrigue believes that bacterial conjugation offers more versatility than phage delivery: »While phages are limited in the amount of DNA they can carry, conjugation doesn't have such constraints. Moreover, phages have a limited host range – which can be a drawback for comprehensive infection treatment - and resistance can develop more swiftly,« he says.
He also emphasises that there are ways to ensure that the conjugation system doesn’t linger, thereby decreasing the time that gene editing is active and reducing the risk of off-target edits. »One strategy is to insert mutations in the donor strain, making it dependent on a specific molecule that isn't naturally present in the microbiota. Additionally, we've engineered conjugative plasmids that only transfer the CRISPR system and not itself, so the introduced plasmid can't replicate in the recipient bacteria,« explains Rodrique.
Want to learn more about delivery systems?
Read our previous articles about gene-editing delivery systems and find relevant webinars right here.
References
- Chiesa R, Georgiadis C, Syed F, et al. Base-Edited CAR T Group. Base-Edited CAR7 T Cells for Relapsed T-Cell Acute Lymphoblastic Leukemia. N Engl J Med. 2023 Sep 7;389(10):899-910.
- Rosenblum D, Gutkin A, Kedmi R, et al. CRISPR-Cas9 genome editing using targeted lipid nanoparticles for cancer therapy. Sci Adv. 2020 Nov 18;6(47):eabc9450.
- Qiu M, Glass Z, Chen J, et al. (2021) Lipid nanoparticle-mediated codelivery of Cas9 mRNA and single-guide RNA achieves liver-specific in vivo genome editing of Angptl3. Proc Natl Acad Sci USA. 2021 March 1;118(10): e2020401118
- Cheng Q, Wei T, Farbiak L, et al. Selective organ targeting (SORT) nanoparticles for tissue-specific mRNA delivery and CRISPR-Cas gene editing. Nat Nanotechnol. 2020 Apr;15(4):313-320.
- Kasiewicz LN, Biswas S, Beach A, et al. GalNAc-Lipid nanoparticles enable non-LDLR dependent hepatic delivery of a CRISPR base editing therapy. Nat Commun. 2023 May 15;14(1):2776.
- Zou Y, Sun X, Yang Q, et al. Blood-brain barrier-penetrating single CRISPR-Cas9 nanocapsules for effective and safe glioblastoma gene therapy. Sci Adv. 2022 Apr 22;8(16):eabm8011.
- Vera LNP, Schuh RS, Fachel FNS, et al. Brain and visceral gene editing of mucopolysaccharidosis I mice by nasal delivery of the CRISPR/Cas9 system. J Gene Med. 2022 Apr;24(4):e3410.
- Gencay YE, Jasinskytė D, Robert C, et al. Engineered phage with antibacterial CRISPR-Cas selectively reduce E. coli burden in mice. Nat Biotechnol. 2023 May 4.
- Neil K, Allard N, Roy P, et al. High-efficiency delivery of CRISPR-Cas9 by engineered probiotics enables precise microbiome editing. Mol Syst Biol. 2021 Oct;17(10):e10335.
- Allard N, Collette A, Paquette J, et al. Systematic investigation of recipient cell genetic requirements reveals important surface receptors for conjugative transfer of IncI2 plasmids. Commun Biol. 2023 Nov 16;6(1):1172.
Other useful resources
Madigan V, Zhang F, Dahlman JE. Drug delivery systems for CRISPR-based genome editors. Nat Rev Drug Discov. 2023 September 18;22(875)
To get more CRISPR Medicine News delivered to your inbox, sign up to the free weekly CMN Newsletter here.
Tags
ArticleInterviewNewsDeliveryElectroporationLipid-based nanoparticleRibonucleoprotein (RNP)Adeno-associated virus (AAV)Adenovirus (AV)Lentivirus (LV)Epigenome editing (e-GE)Intellia Therapeutics, Inc.Verve Therapeutics, Inc.
CLINICAL TRIALS
Sponsors:
Suzhou Maximum Bio-tech Co., Ltd.
Sponsors:
Zhejiang University